Anastasiia Stepanova | CELLS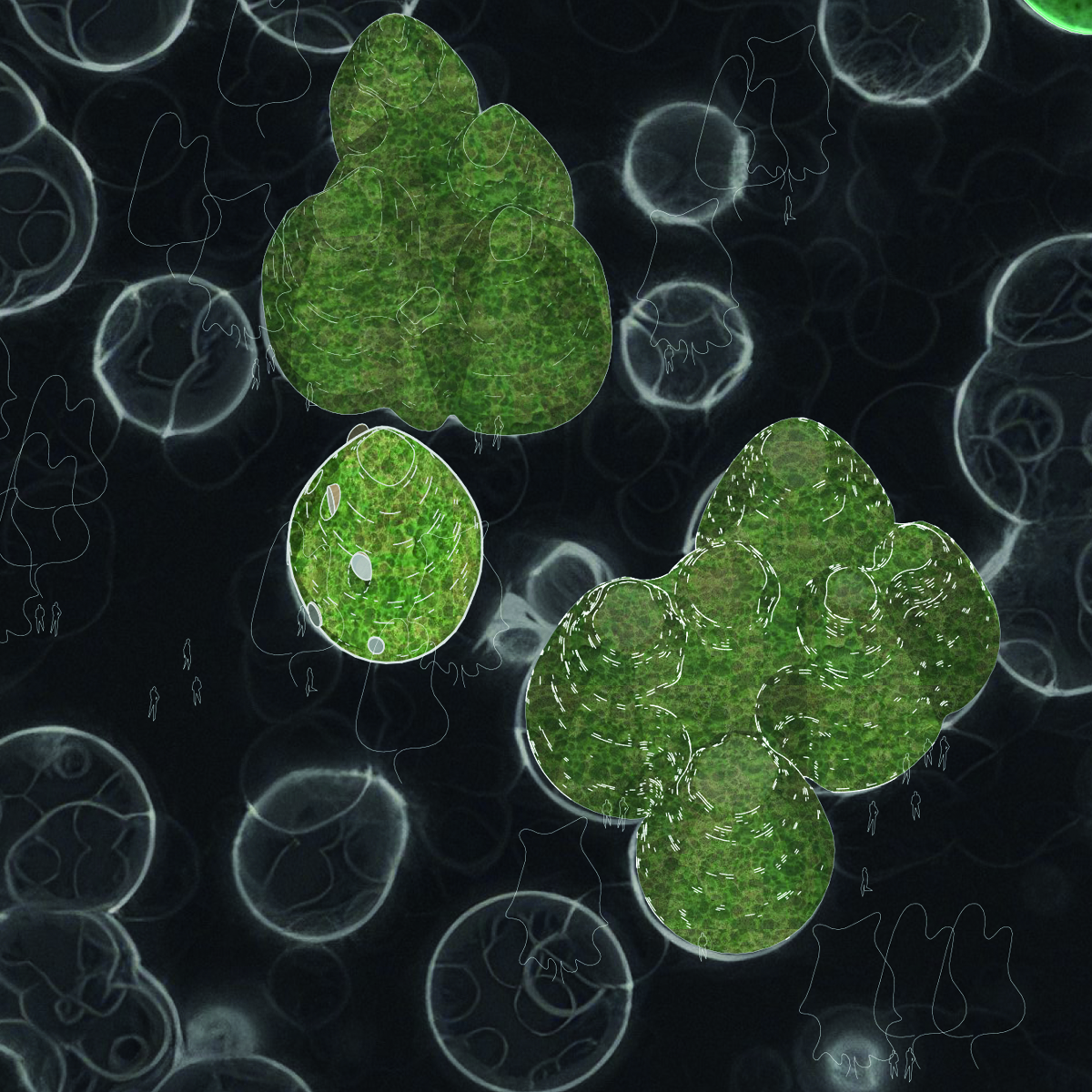
Production of cement significantly impacts the environment, both through emissions into the atmosphere and the use of natural resources. Some key environmental impacts of cement production include:
□ Carbon Dioxide Emissions (CO2): Cement production is one of the largest sources of anthropogenic CO2 emissions. The primary source of CO2 is limestone, which, during the burning process, transforms into calcium oxide (CaO), releasing carbon dioxide.
□ Emissions of Other Harmful Substances: Various pollutants, such as nitrogen oxides (NOx), sulfur dioxide (SO2), and particulate matter, are released into the air during the cement burning process, negatively affecting human health and the environment.
□ Use of Natural Resources: Mining raw materials for cement production, such as limestone and clay, can lead to the exploitation of natural resources and degradation of ecosystems.
□ Waste and Outputs during the Process: The burning and other production steps generate waste materials, including dust, sludge, and other substances, which can negatively impact water systems and soil.
□ Energy Consumption: Cement burning requires a substantial amount of energy, and some of it may come from low-carbon sources like coal.
To reduce the environmental impact of cement production and enhance sustainability, the industry explores and implements various technologies and methods, including renewable energy sources, improved burning processes, efficient raw material use, and waste recycling. These innovations aim to reduce greenhouse gas emissions and mitigate negative environmental impacts.
Chemical equations for the creation of Portland cement involve burning limestone and clay in a rotary kiln at high temperatures. Various chemical reactions occur during burning, forming the basic components of cement.
Calcium oxide (CaO), or lime, also known as uncalcined limestone, can be obtained by calcining (heating) limestone (CaCO3) at high temperatures. This process, called calcination, is chemically described by the following reaction:
CaCO3 → CaO + CO2
In this equation, limestone decomposes at high temperatures into calcium oxide and carbon dioxide. This process occurs at temperatures above 825 degrees Celsius (1500 degrees Fahrenheit).
PRIMARY COMPONENTS OF CEMENT
Tricalcium Silicate:
Chemical formula: 3CaO * SiO2
Provides strength and hardness to concrete.
Dicalcium Silicate:
Chemical formula: 2CaO * SiO2
Provides additional strength and influences the setting time of cement.
Tricalcium Aluminate:
Chemical formula: 3CaO * Al2O3
Influences early strength and participates in the setting process.
Tetracalcium Aluminoferrite:
Chemical formula: 4CaO * Al2O3 * Fe2O3
Participates in the formation of strength and color of cement.
CaCO3 + SiO2 + Al2O3 + Fe2O3 → Ca3SiO5 + Ca2SiO4 + Ca3Al2O6 + Ca4Al2Fe2O10 + CO2
Limestone (CaCO3), silica (SiO2), clay (Al2O3), and iron oxide (Fe2O3) are burned in a rotary kiln. The result of chemical reactions is the basic components of cement: tricalcium silicate (C3S), dicalcium silicate (C2S), tricalcium aluminate (C3A), and tetracalcium aluminoferrite (C4AF).
Carbon dioxide (CO2) is released. Producing 1.0 kg of cement generates 0.6 kg of CO2 emissions.
Cyanobacteria, also known as blue-green algae, represent a diverse group of bacteria capable of photosynthesis. Their distinguishing feature compared to other bacteria is the presence of chlorophyll a pigment used to capture light energy during photosynthesis, giving them their blue-green color.
Cyanobacteria can be unicellular, filamentous (forming chains), or colonial (forming colonies of several cells).
They can be utilized in various applications, including biocement production. Biocement is a construction material where biological processes contribute to material hardening. The use of cyanobacteria in biocement creation can improve ecological and structural properties.
Here are some ways cyanobacteria can be used in biocement production:
Carbonation: Cyanobacteria can stimulate the carbonation process, converting atmospheric carbon dioxide into calcium carbonate. This process can lead to gradual concrete strengthening over time.
Biocement Production: Cyanobacteria can release calcium carbonate during their growth. This carbonate can be used as a major component in biocement creation, providing a more environmentally friendly alternative to traditional cement.
Formation of Biological Aggregates: Cyanobacteria can be used to create biological aggregates in the concrete matrix. These aggregates can enhance mechanical properties such as strength and crack resistance.
Improvement of Environmental Sustainability: The use of cyanobacteria can reduce the environmental impact of concrete production since cyanobacteria can fix carbon dioxide.
However, despite potential benefits, the use of cyanobacteria in biocement faces technical and technological challenges, such as controlling cyanobacteria growth and ensuring process stability.
Currently, there is no specific type of cyanobacteria unequivocally identified as the best for biocement formation. Research in this field continues to develop, and different cyanobacteria species may have varying effectiveness under different conditions.
Nevertheless, different types of cyanobacteria and their potential advantages can be considered:
Unicellular Cyanobacteria: Simple Control: Unicellular cyanobacteria may be easier to control in biocement production conditions.
Filamentous Cyanobacteria: Structural Formation: Filamentous cyanobacteria can form long filaments, which may be useful for creating a stronger and more durable structure in the concrete matrix.
Colonial Cyanobacteria: Colony Formation: Colonial cyanobacteria can form colonies, which can also impact the mechanical properties of biocement.
Can Cyanobacteria be directed to build concrete in a specific direction? Yes, it requires a combination of biological and engineering approaches.
Engineering Constructs:
Matrix Development: Creating a concrete matrix that provides support and direction for cyanobacteria growth. The matrix must be strong and capable of retaining the calcium carbonate released by cyanobacteria.
Use of Molds: Using molds or specific surfaces that serve as guides for cyanobacteria growth and concrete formation.
Selection of Suitable Cyanobacteria:
Genetic Modification: Exploring the possibility of genetically modifying cyanobacteria to enhance their carbonate-forming abilities and adapt to specific conditions.
Control of Growth Conditions:
Optimization of Conditions: Creating conditions for photosynthesis and carbonate formation, requiring control over lighting, temperature, pH, and nutrients in the system.
Monitoring and Regulation: Utilizing automated monitoring systems to track and regulate growth parameters.
Biomimicry:
Mimicking Natural Processes: Studying natural conditions under which cyanobacteria build structures and creating concrete environments that mimic these conditions.
Innovative Construction Methods:
Bioprinting: Advancing bioprinting technologies for precise control of cyanobacteria distribution in three-dimensional space.
Forming Methods: Using forming and growth direction methods to create desired shapes and structures. |
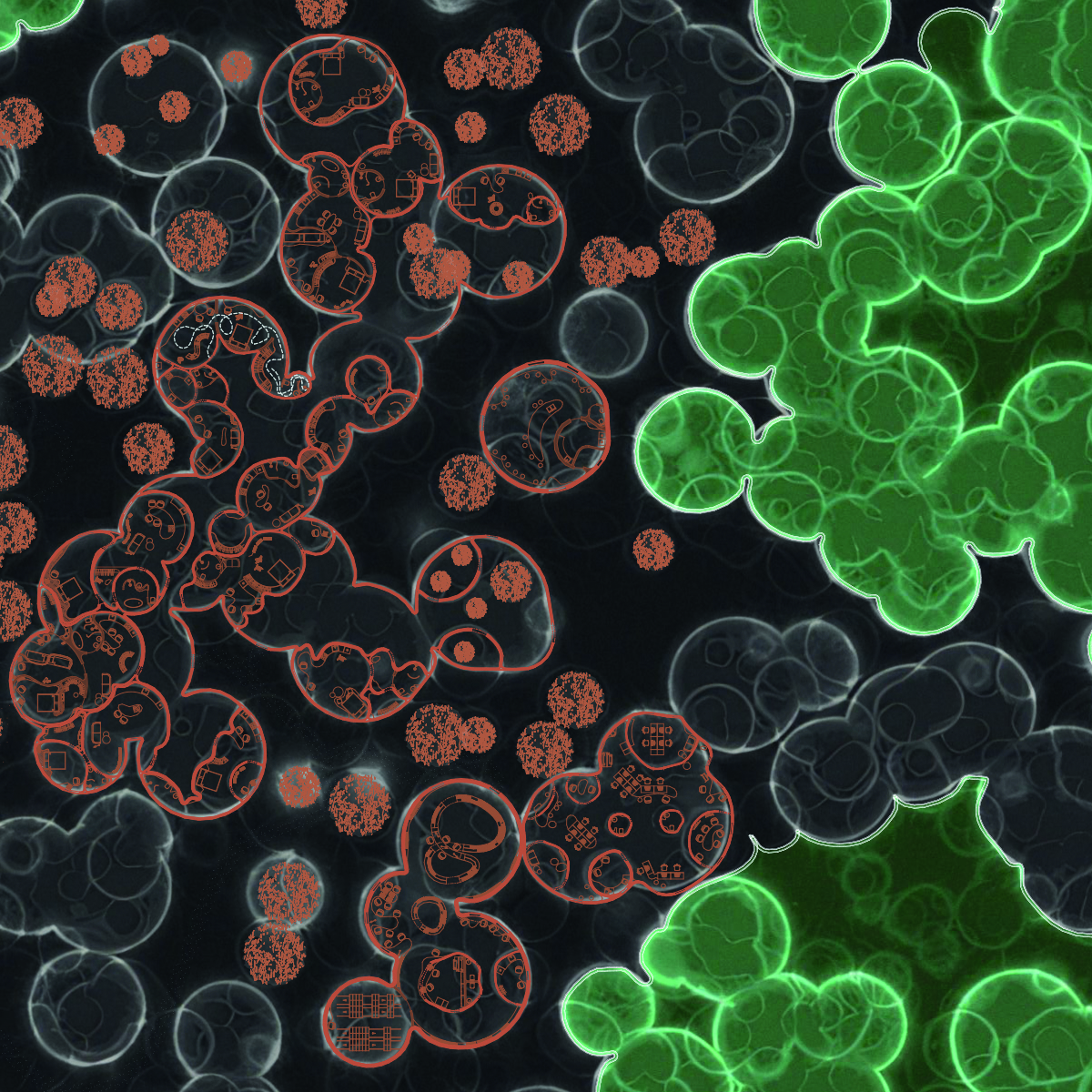
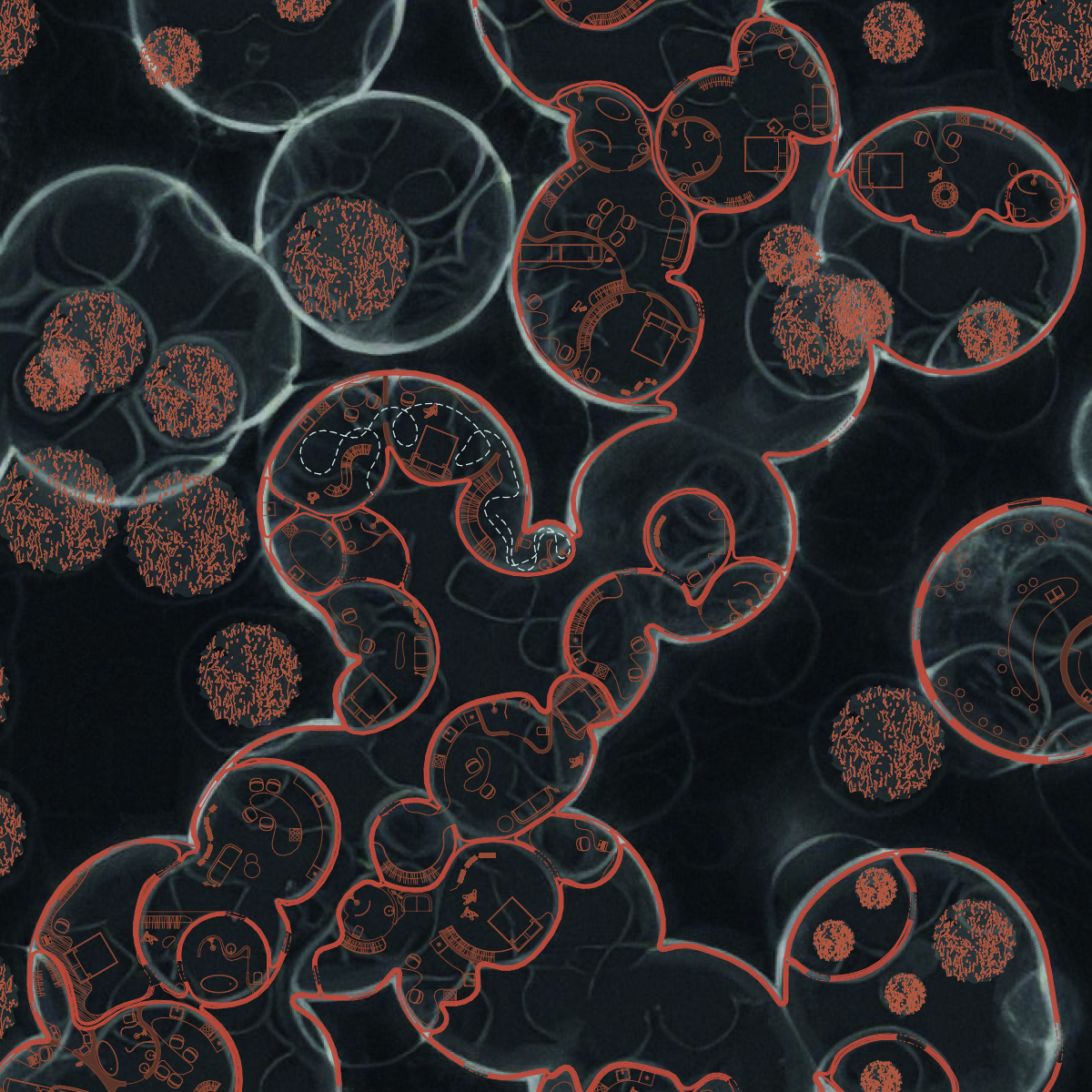
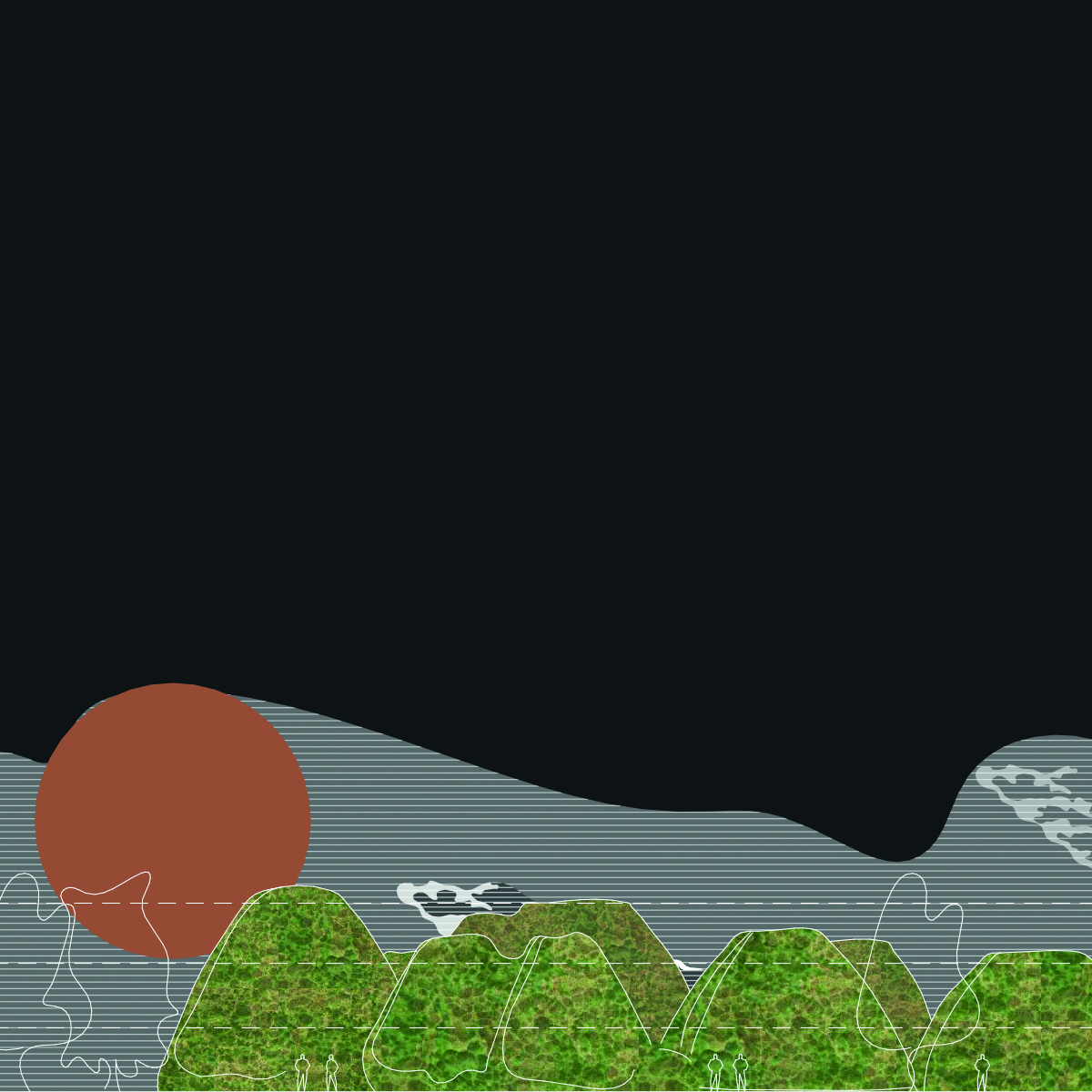
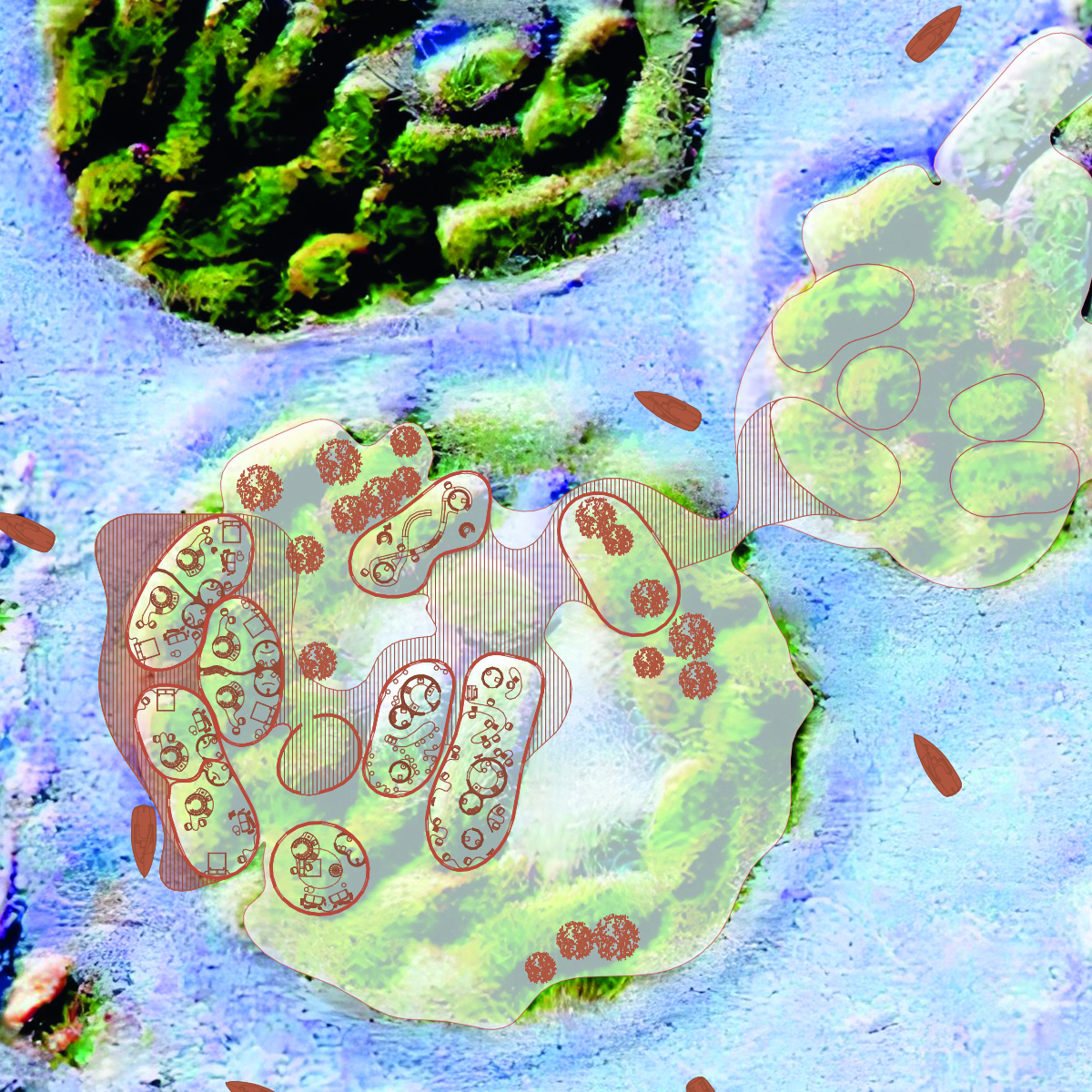
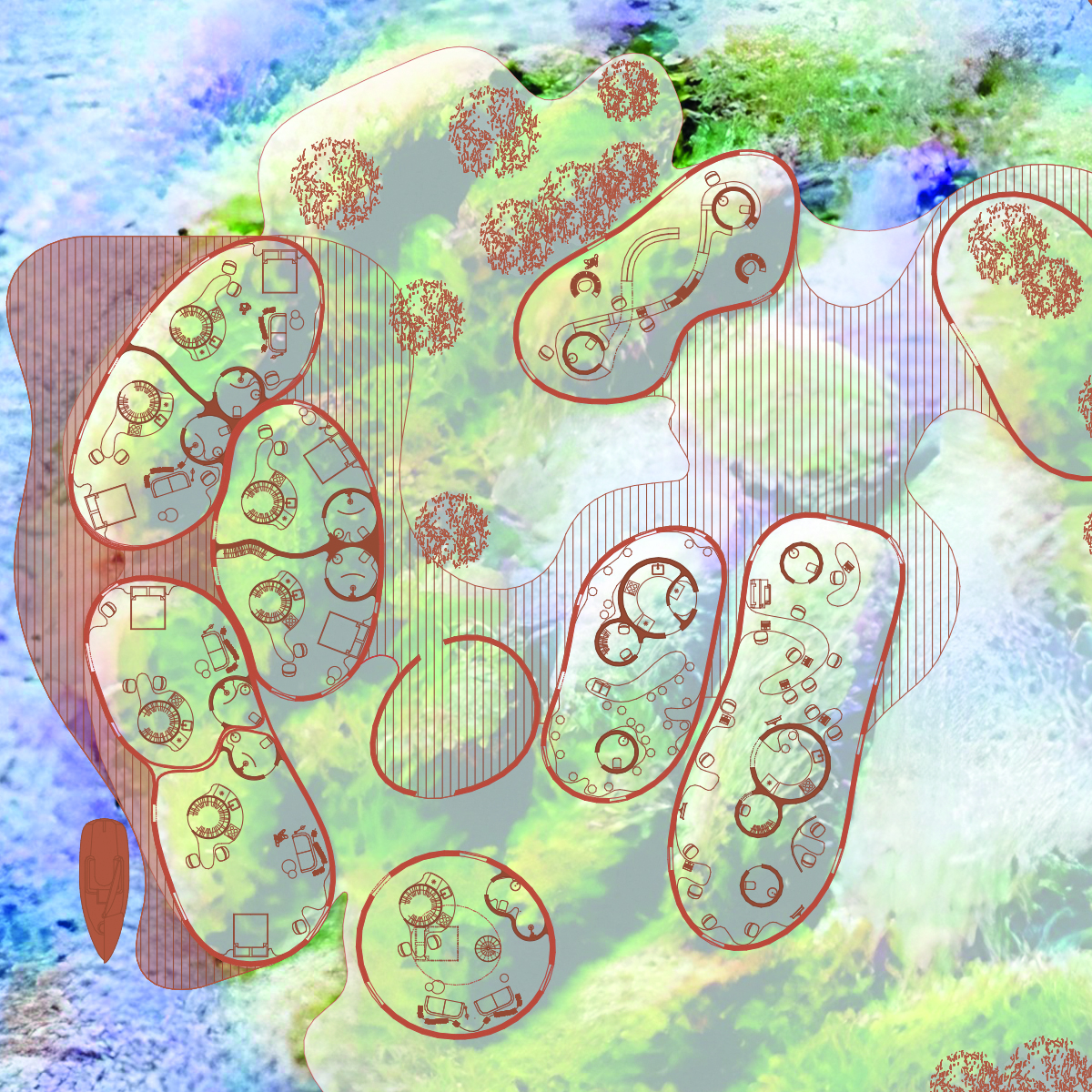
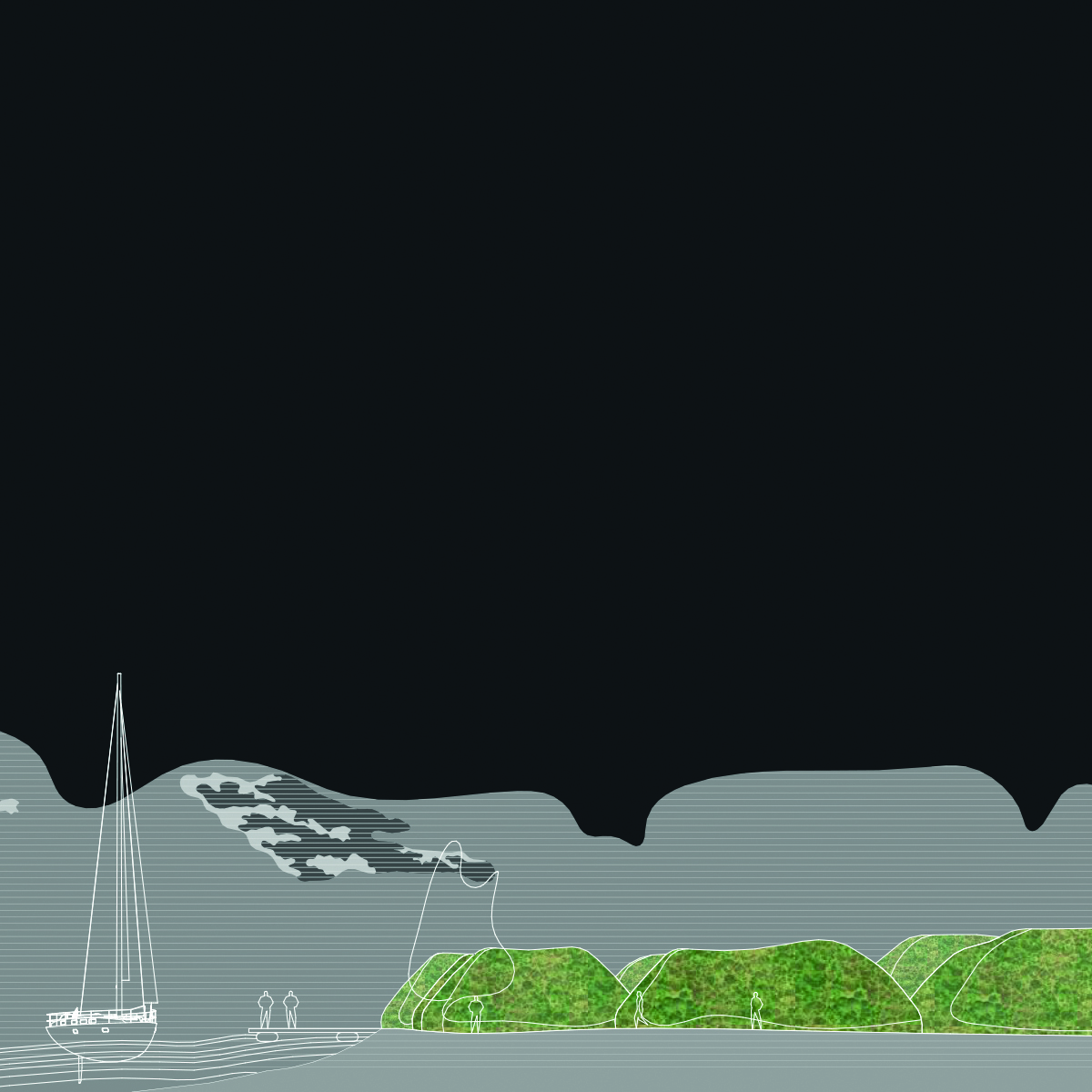
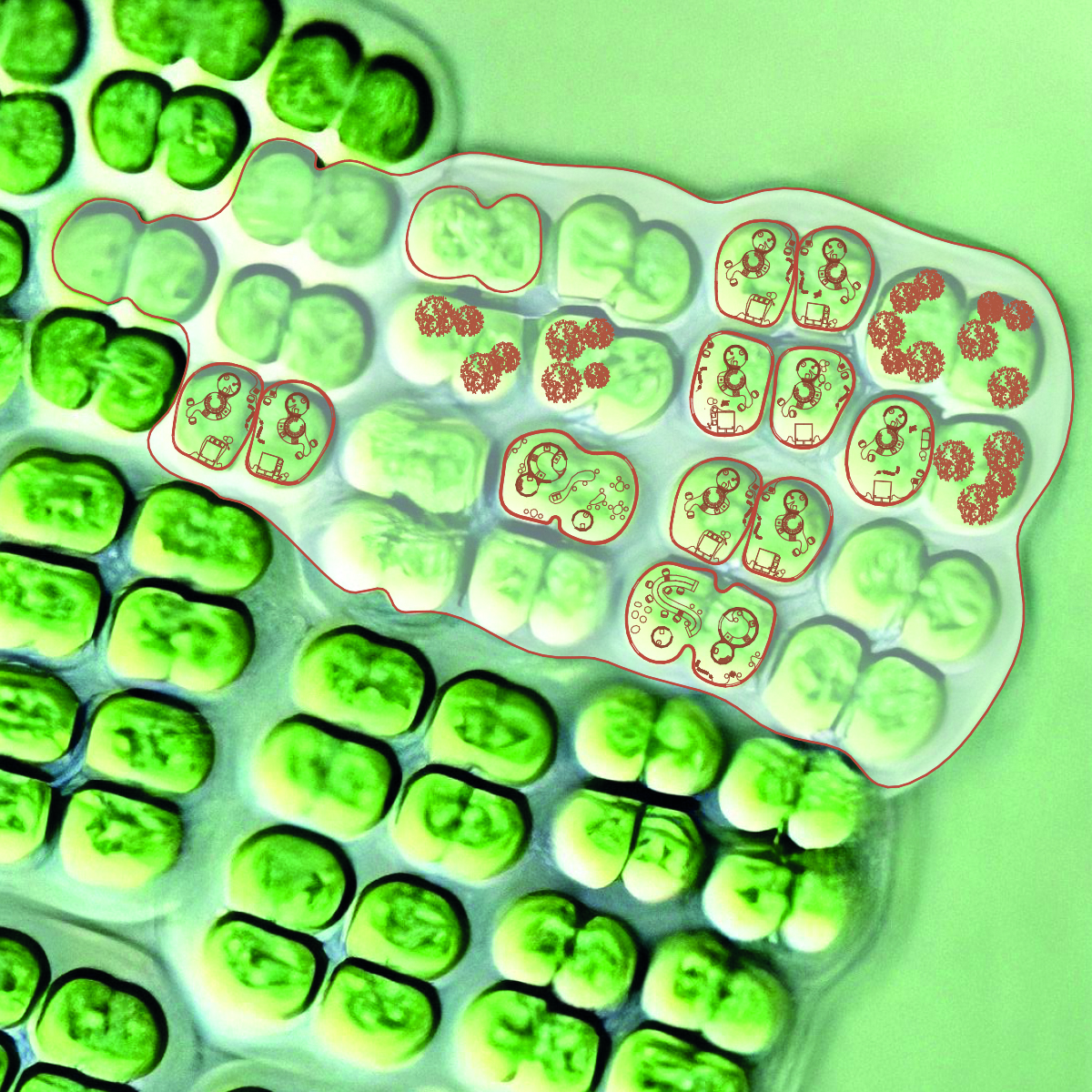
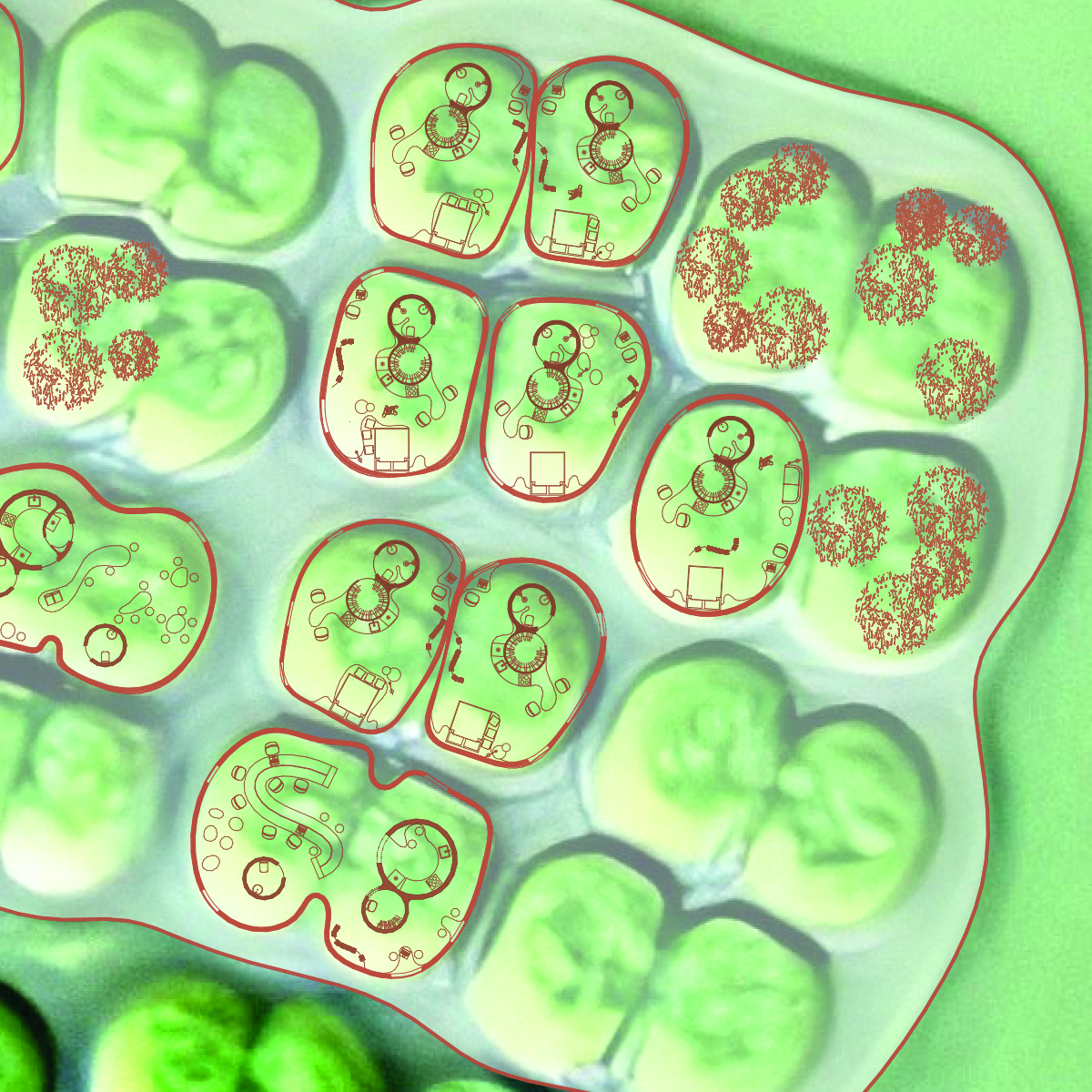
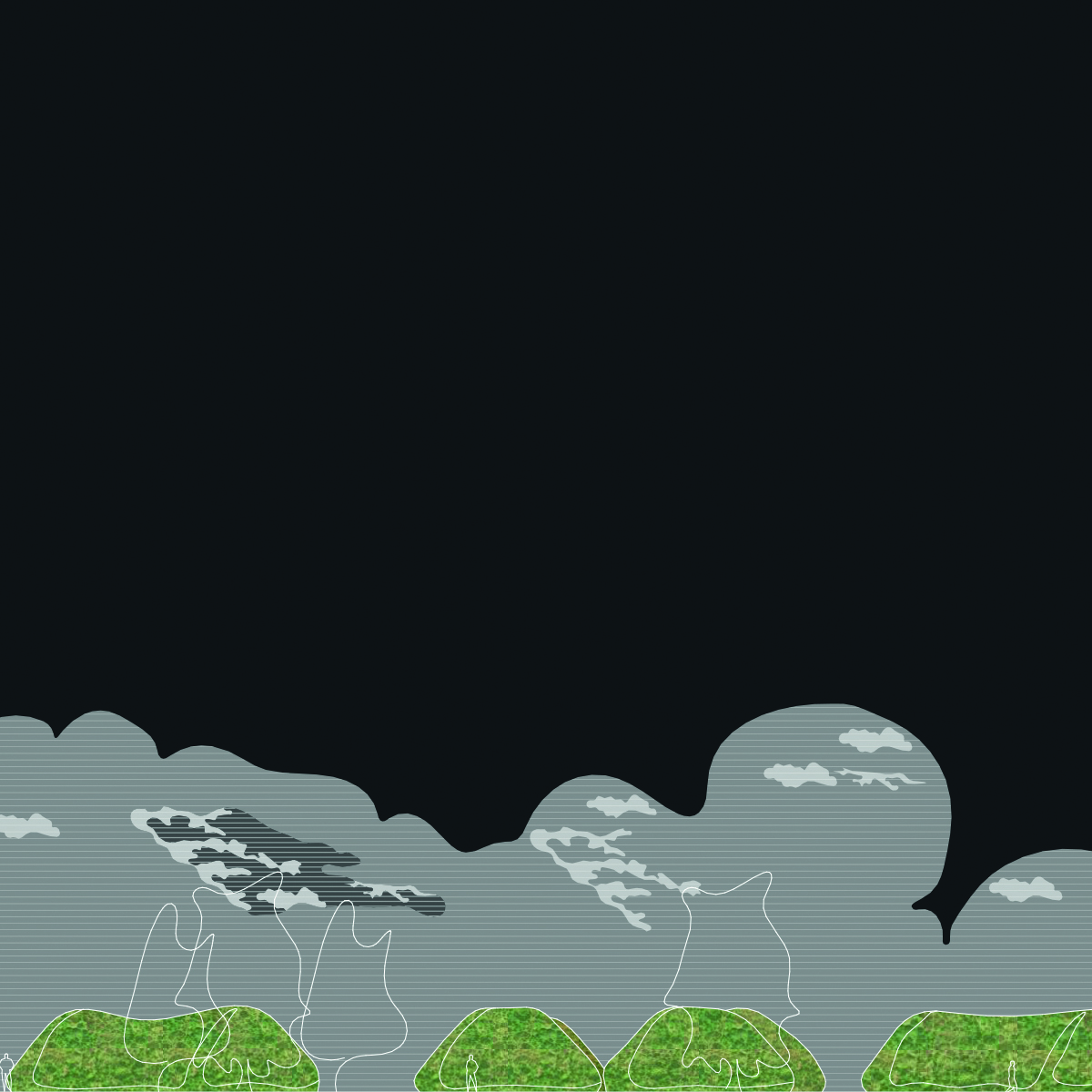
|